10 Devices to detect and monitor radiation
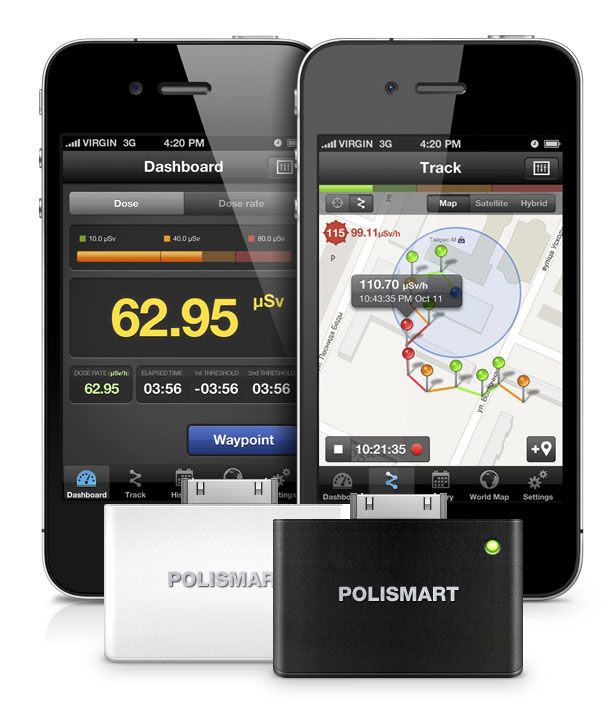
Detecting and Measuring Ionizing Radiation
Since ionizing radiation is invisible and undetectable by human senses, innovative technologies have been developed to detect and measure this type of radiation effectively. These advancements include a variety of devices tailored to specific radiation sources and monitoring needs. Modern dosimeters, in particular, have evolved significantly, incorporating features that enhance both user safety and data management.
Modern Dosimeters: Enhancing Safety and Data Management
Today’s dosimeters are often equipped with advanced capabilities, including:
- Self-Reading Capabilities: Dosimeters now feature digital displays that provide instant, easy-to-read access to radiation exposure data.
- Alarm Systems: These devices come with alarms that notify users of real-time radiation exposure levels, providing urgent warnings when levels spike unexpectedly, ensuring immediate awareness and enhancing safety in potentially hazardous environments.
“Modern dosimeters provide instant access to radiation data and real-time alerts, enhancing user safety and decision-making in critical situations.”
Connectivity and Comprehensive Data Management
Further enhancing their utility, many modern dosimeters can connect electronically to databases and software programs. This connectivity offers several advantages:
- Remote Monitoring: Health and safety managers can monitor radiation exposure across teams and environments.
- Regulatory Compliance: Accurate records are maintained for compliance with safety regulations.
- Customizable Settings: Device settings can be adjusted remotely to meet specific safety protocols.
Example of Advanced Technology: Radiation Detector PM1904 for iPhone
An example of such advanced technology is the Radiation Detector PM1904 for iPhone, developed by Polimaster Inc. This compact, innovative pocket dosimeter integrates seamlessly with smartphones to offer convenient radiation monitoring on the go. Key features include:
- User-Friendly Interface: Easy to use with smartphones.
- LED Light Alarms: Provides alerts when preset radiation thresholds are exceeded.
- Secure Data Storage: All exposure measurements are stored in non-volatile memory, preserving data integrity even in power disruptions.
For more information on understanding ionizing radiation and protection, visit Understanding Ionizing Radiation and Protection.
1. Optically Stimulated Luminescence (OSL) Detectors
Optically Stimulated Luminescence (OSL) detectors, such as the widely recognized Luxel dosimeter, play a crucial role in radiation monitoring across various sectors, including healthcare and industrial safety. Utilizing advanced OSL technology, these devices are particularly favored for their precision and reliability in detecting radiation exposure.
How OSL Detectors Work
The core of a Luxel dosimeter is a radiation-sensitive layer made of aluminum oxide, which is hermetically sealed within a packet resistant to light and moisture. When exposed to radiation, electrons within the aluminum oxide become trapped in an elevated energy state. These electrons remain trapped until stimulated by a laser emitting a specific wavelength of light. This interaction frees the electrons, allowing them to release their stored energy as visible light. The intensity of this light is directly proportional to the amount of radiation absorbed by the dosimeter, enabling precise measurement of the exposure.
“The intensity of the visible light released by the Luxel dosimeter is directly proportional to the amount of radiation absorbed, allowing for precise measurement.”
Accuracy and Sensitivity
To enhance measurement accuracy, the Luxel dosimeter includes a series of optical filters. These filters allow the device to:
- Quantify the amount of radiation.
- Determine the specific type of radiation (such as X-rays, gamma rays, or beta radiation).
- Identify the energy levels involved.
For optimal performance and accurate directional measurement, it is crucial that the dosimeter is oriented correctly, with the front facing towards the radiation source. This positioning ensures that the readings reflect the actual exposure levels experienced by the wearer.
Applications and Sensitivity
OSL detectors like the Luxel are highly valued for their ability to detect very low levels of radiation:
- As little as 1 millirem for X-rays and gamma rays.
- 10 millirem for more penetrating forms of radiation like energetic beta rays.
Their high sensitivity makes them indispensable tools for ensuring safety in environments where even minimal radiation exposure must be monitored and controlled.
For further insights into radiation monitoring technologies, explore Understanding Ionizing Radiation and Protection.
For more information on radiation detection technologies and safety protocols, visit Understanding Ionizing Radiation and Protection.
2. Thermoluminescent Dosimeters (TLDs)
Thermoluminescent Dosimeters, commonly referred to as TLDs, serve as a sophisticated alternative to traditional film badges in the field of radiation monitoring. These devices are typically worn by individuals exposed to ionizing radiation for a period, generally not exceeding three months, after which they require processing to assess the accumulated radiation dose.
How TLDs Work
At the core of a TLD lies a phosphor material embedded within a solid crystal structure. When exposed to ionizing radiation, this phosphor absorbs the energy and traps electrons. Upon heating, the TLD releases these electrons, causing the phosphor to emit light—a phenomenon directly proportional to the radiation dose received. This emitted light is meticulously measured to provide an accurate quantification of radiation exposure.
“When heated, the TLD releases trapped electrons, causing the phosphor to emit light proportional to the radiation dose received.”
Common Phosphor Materials
The most commonly used phosphor materials in TLDs include:
- Calcium Fluoride: Sensitive predominantly to gamma rays, making it ideal for gamma radiation monitoring.
- Lithium Fluoride: Sensitive to both gamma rays and neutrons, offering a broader scope of radiation detection.
Advantages of TLDs
TLDs are highly prized for their precision and sensitivity to low levels of radiation, capabilities that are approximately equivalent to those of film badges under routine conditions. They can detect radiation doses as minute as 1 millirem. Unlike film badges, TLDs can be reused, which provides a cost-effective solution for ongoing radiation monitoring.
Limitations of TLDs
However, it is important to note that TLDs:
- Do not provide an immediate readout of radiation exposure.
- Cannot be read multiple times for the same exposure period, limiting their use in situations requiring instant data retrieval or continuous monitoring.
Applications of TLDs
The versatility of TLDs makes them particularly valuable in diverse applications, including:
- Environmental monitoring
- Ensuring the safety of personnel in radiation-prone work areas
- Medical and scientific settings
Their ability to accurately measure and track radiation exposure with minimal user intervention makes TLDs indispensable in many industrial, medical, and scientific environments.
For more information on radiation monitoring technologies, explore Understanding Ionizing Radiation and Protection.
3. Film Badges
Film badges have been a longstanding tool for radiation monitoring, particularly among X-ray technicians. These badges use a piece of radiation-sensitive film enclosed in a light-proof envelope to capture exposure data over the badge’s wear time. Exposed to gamma rays, X-rays, and beta particles, the film undergoes a change that correlates with the amount of radiation absorbed—the more significant the exposure, the greater the visible change upon development.
Film Badges for Radiation Monitoring
Film badges have long been a preferred choice for personnel radiation monitoring due to their cost-effectiveness and ability to provide a permanent record of radiation exposure. Typically worn between the collar and waist, these badges use radiation-sensitive film to capture exposure data over a specific period, ensuring accurate measurement of radiation dose in the wearer’s environment.
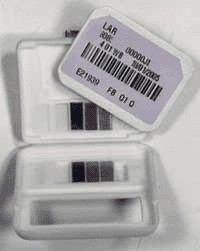
Advantages of Film Badges
- Affordability: Film badges are relatively inexpensive, making them accessible for widespread use in various settings, including hospitals and industrial facilities.
- Permanent Record: The film in these badges provides a lasting record of radiation exposure, which is essential for compliance and long-term health monitoring.
Drawbacks of Film Badges
- Development Delays: Film badges must be developed in a lab, which can delay obtaining exposure results.
- Sensitivity to Conditions: High temperatures and other environmental factors can degrade the film, leading to inaccurate readings.
- Low Sensitivity: These badges are generally unable to measure radiation exposures below 20 millirem of gamma radiation effectively.
Despite these drawbacks, film badges remain a valuable tool in radiation monitoring. Their affordability and ability to provide a permanent record make them indispensable in many occupational settings.
For more information on radiation monitoring and safety protocols, explore our resources on Radiation Dose in Modern Diagnostic Radiology.
Proper Usage and Storage
Proper usage and storage are crucial for accurate functioning. When not in use, it is recommended to store film badges in a radiation-free environment to prevent unintended exposure that could lead to false readings. In cases of overexposure, thorough investigations are typically conducted to determine whether the exposure was accidental or the result of improper handling, underscoring the importance of careful and correct usage of these monitoring devices.
Common Causes of Film Badge Overexposure
- Purposeful exposure of the film badge: Intentional exposure to test the badge’s response or to manipulate readings can lead to inaccurate data.
- Improper film badge storage: Storing badges in areas where they can be inadvertently exposed to radiation or environmental factors that could affect their sensitivity.
- Failure to use protective shielding: Not utilizing proper shielding can result in higher than expected exposure readings.
- Inappropriate working techniques: Techniques that expose the badge to radiation more than necessary can skew results.
- Insufficient or faulty radiation shielding: Inadequate shielding at workplaces can lead to excessive exposure recorded by the badge.
- Wearing the film badge unintentionally during medical procedures: Accidentally wearing the badge during diagnostic or therapeutic X-rays can cause significant overexposures.
- Failure to recognize a film packet used for non-routine recording: Not identifying or mismanaging a film badge that has been designated for specific testing or non-regular use.
For more detailed information on radiation monitoring and safety protocols, explore the resources available at Radiation Dose in Modern Diagnostic Radiology.
4. Pocket Dosimeters
Pocket dosimeters provide real-time, immediate feedback on radiation exposure, making them critical for professionals working in high-risk environments. These devices are especially valuable in industrial radiography and other settings where exposure levels can fluctuate rapidly. There are two main types commonly used in these settings: the Direct Read Pocket Dosimeter and the Digital Electronic Dosimeter.
Direct Read Pocket Dosimeters
Direct Read Pocket Dosimeters are designed for quick and straightforward checks of radiation levels. Their simple, robust construction allows for easy use and reusability. Key features include:
- Quick Readings: Provides immediate feedback on radiation exposure, allowing for on-the-spot decisions.
- Reusability: These dosimeters can be reset for multiple uses, making them cost-effective.
Digital Electronic Dosimeters
Digital Electronic Dosimeters offer more detailed information and advanced features. They are particularly useful for continuous monitoring and detailed record-keeping. Key features include:
- Detailed Information: Displays dose rate and total accumulated dose, providing comprehensive data.
- Digital Displays: Easy-to-read digital screens ensure quick and accurate interpretation of data.
- Alarms: Built-in alarms warn users of high radiation levels, enhancing safety.
For more detailed information on how pocket dosimeters can enhance safety in high-risk environments, visit our resource on Radiation Monitoring.
Limitations and Advantages of Pocket Dosimeters
Pocket dosimeters are highly portable and convenient for real-time radiation monitoring, yet they come with certain limitations. One notable drawback is their limited measurement range, which may not be adequate in high-radiation environments. Additionally, they do not provide a permanent record of radiation data, which is essential for long-term monitoring and analysis. The physical design of these dosimeters also makes them prone to damage from drops or impacts, potentially leading to data loss at critical moments.
Advantages of Digital Electronic Dosimeters
Despite these limitations, digital electronic dosimeters, a common type of pocket dosimeter, offer several compelling advantages:
- Geiger-Müller Counters: These devices use Geiger-Müller counters to detect and measure radiation exposure accurately.
- Electronic System: The built-in electronic system collects charge generated by detected radiation. Once a preset threshold is met, the charge is discharged, activating an electronic counter that calculates and displays the accumulated dose and dose rate.
- Safety Features: Many models are equipped with audible alarms that alert users when certain radiation exposure levels are exceeded, providing immediate warnings and enhancing user safety.
For more detailed information on the features and benefits of digital electronic dosimeters, visit our resource on Digital Dosimeters.
5. Scintillators: Essential Tools in Radiation Detection
Scintillators are specialized materials extensively used in radiation detection due to their unique ability to luminesce when exposed to ionizing radiation. These materials absorb energy from incoming particles and then reemit that energy as light. This process involves the scintillator material entering an excited state. Depending on the composition, the return to a stable state—and thus the emission of light—can range from almost instantaneous to several hours.
How Scintillators Work
Scintillators function by absorbing radiation energy and converting it into visible light. This conversion is crucial for detecting and measuring radiation levels accurately. The emitted light is typically captured by photomultiplier tubes (PMTs) or photodiodes, which convert the light into electrical signals for measurement and analysis.
Applications of Scintillators
Due to their precise timing and sensitivity, scintillators are used in various applications:
- Medical Imaging: Technologies like PET (Positron Emission Tomography) and CT (Computed Tomography) scans use scintillators to produce clear and accurate images by detecting radiation from tracer isotopes or X-rays.
- Nuclear Physics: Scintillators are essential in nuclear physics experiments for detecting and measuring radiation from nuclear reactions and decay processes.
- Environmental Monitoring: These materials are employed in radiation detectors to monitor environmental radiation levels, ensuring public safety and regulatory compliance.
Customizing Scintillator Properties
Scintillator properties can be fine-tuned based on the material composition to optimize their performance for specific applications. Various materials like sodium iodide (NaI), bismuth germanate (BGO), and lutetium oxyorthosilicate (LSO) are chosen for their specific light output, decay time, and radiation absorption efficiency. This customization allows for the development of highly specialized radiation detection systems tailored to the needs of different industries.
For more detailed information on scintillator types and applications, visit our resource on Scintillator Materials.
Applications of Scintillators in Various Fields
Scintillators are versatile materials employed in a range of critical applications across multiple sectors. Here are some key areas where scintillators play a pivotal role:
Homeland Security
Utilized by the American government, scintillators are essential components in Homeland Security’s radiation detection systems. They help ensure national safety by identifying radioactive materials. For more information on radiation detection in security, visit Homeland Security Radiation Detection.
Scientific Research
In scientific research, scintillators are crucial for neutron and high-energy particle physics experiments where precise detection of radiation is essential. They enable researchers to study fundamental particles and forces, advancing our understanding of the universe.
Energy Sector
Scintillators play an instrumental role in the energy sector, aiding in the exploration of new resources. They are used in X-ray security systems and nuclear cameras to identify and analyze materials. In gas and oil exploration, scintillators provide detailed internal images, supporting the detection of reserves. Learn more about their use in advanced imaging techniques like computed tomography and laser mammography.
Medical Diagnostics
Medical diagnostics benefit significantly from scintillators. They are integral to CT scanners and gamma cameras, enabling detailed internal views crucial for accurate diagnosis. Their precision and sensitivity help in identifying and treating medical conditions effectively.
Everyday Technology
Scintillators also enhance everyday technology. They improve the quality of images on computer monitors and television sets by converting ionizing radiation into visible light. This application is critical in delivering high-definition visual experiences.
Nuclear Safety
In nuclear safety, scintillators monitor and detect changes in radiation levels, indicating potential instability or leakage. Their reliability ensures the safe management of nuclear materials.
Energy-Efficient Lighting
Scintillators contribute to energy-efficient lighting solutions. They generate light in fluorescent tubes, demonstrating their utility in providing bright, efficient illumination. For more on energy-efficient lighting, visit Energy Efficient Lighting.
Scintillators are indispensable in these diverse applications, showcasing their versatility and importance in modern technology and safety. Their ability to detect and convert radiation into usable data or light makes them invaluable across numerous fields.
6. Ionization Chambers and Proportional Counters
Ionization Chambers: Essential Tools in Radiation Detection
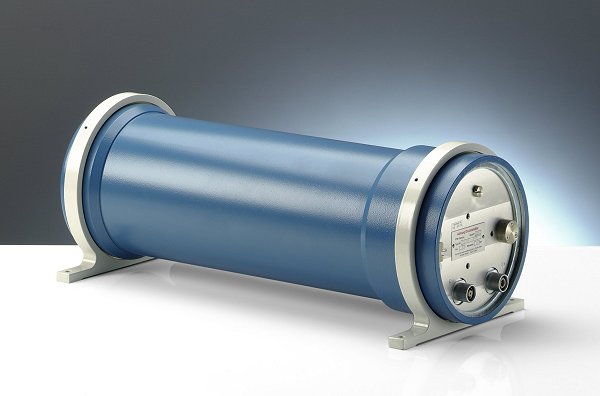
Ionization chambers are fundamental tools in radiation detection, known for their simplicity and effectiveness. These gas-filled radiation detectors are used across various sectors to detect and measure ionizing radiation, such as X-rays, gamma rays, and beta particles. They operate by measuring the electric charge created by ionized gas within the chamber as radiation passes through, providing a direct and reliable method for assessing radiation levels.
Uniform Response Across Radiation Energies
Ionization chambers are particularly noted for their uniform response to a wide range of radiation energies, making them exceptionally versatile and dependable for quantitative assessments. They are especially favored for accurately measuring high levels of gamma radiation, which is crucial in environments with significant radiation exposure.
Applications of Ionization Chambers
The broad application of ionization chambers spans several critical fields, including:
- Nuclear Power Industry: Used for monitoring and safety protocols to ensure safe radiation levels.
- Research Laboratories: Provide precise radiation measurement essential for experimental integrity.
- Medical Radiography: Ensure safe levels of exposure in diagnostic imaging and treatment.
- Environmental Monitoring: Assess contamination and ecological impacts to maintain environmental safety.
These applications highlight the integral role of ionization chambers in ensuring radiation safety and compliance in many professional settings. Their reliability and accuracy make them indispensable in monitoring radiation and protecting both people and the environment.
For more detailed information about ionization chambers and their various applications, visit Radiation Detection: Ionization Chambers.
Proportional Counters in Radiation Detection
Proportional counters are sophisticated devices that merge the detection techniques of Geiger-Muller tubes and ionization chambers, creating versatile and sensitive radiation detection instruments. These counters are particularly valuable in settings where large area monitoring is required, such as checking for radioactive contamination on personnel, tools, and clothing.
Design and Functionality
Proportional counters are typically installed as stationary instruments due to the logistical challenges associated with mobilizing the gas supplies necessary for their operation. These counters are specifically designed to optimize the detection of alpha and beta radiation.
- Flat Detection Chamber: The detection chamber is often flat, which helps in enhancing the sensitivity and range of the device. This design allows for efficient monitoring over large areas.
- Wire Detectors: Multiple wire detectors span across the detection chamber, providing a broad and sensitive detection area.
- Detection Windows: The detection windows are usually made from materials like metallized mylar, which form part of the cathode structure. This material choice is crucial for accurate and reliable radiation detection.
- Anode Wire: An intricately routed anode wire weaves through the chamber, effectively dispersing throughout the gas-filled space to maximize detection capabilities. This design ensures that the device can accurately measure radiation levels.
For more details on how proportional counters work and their applications, you can visit Radiation Detection: Proportional Counters.
Advantages of Proportional Counters
One of the key advantages of proportional counters is their ability to discriminate between different types of radiation, specifically alpha and beta particles. This capability is crucial for applications requiring precise differentiation in radiation types, which is essential for accurate monitoring and safety assessments in various environments, including:
- Nuclear Facilities: Ensuring safety and compliance by monitoring radioactive contamination.
- Medical Institutions: Safeguarding against radiation exposure in diagnostic and therapeutic settings.
- Environmental Studies: Assessing contamination levels and ecological impacts.
Proportional counters are invaluable tools for detecting and differentiating between alpha and beta radiation, providing accurate and reliable monitoring for safety and research purposes.
For more detailed information on proportional counters and their applications, visit Radiation Detection: Proportional Counters.
7. Geiger Counters
Geiger counters, formally known as Geiger-Müller counters, are essential instruments in radiation detection. These devices measure ionizing radiation, which is invisible and cannot be detected by human senses. To understand more about ionizing radiation and its implications, visit this detailed resource.
How Geiger Counters Work
Geiger counters operate using a Geiger-Müller tube, which detects radiation through ionization within a low-pressure gas contained inside. Here’s a step-by-step breakdown of the process:
- Ionization Process: When ionizing radiation (alpha particles, beta particles, or gamma rays) passes through the Geiger-Müller tube, it ionizes the gas inside.
- Discharge Detection: This ionization causes a discharge, resulting in a detectable pulse of current.
- Counting Radiation Events: Each pulse corresponds to a radiation event, providing a direct count of particle interactions.
Advantages and Limitations
While Geiger counters are highly effective for detecting the presence of radiation, they have certain limitations:
- Energy Levels: Geiger counters do not provide information about the energy levels of the detected particles; they can only confirm their presence.
- Simplicity and Reliability: Despite their limitations, the simplicity, reliability, and ease of use of Geiger counters have maintained their popularity since their invention in 1908.
Applications of Geiger Counters
Geiger counters are widely used across various fields:
- Health Sciences: Monitoring radiation exposure in medical settings.
- Physics: Research and educational purposes in detecting radioactive materials.
- Industrial Settings: Ensuring safety in environments where radiation is present.
- Geology: Detecting naturally occurring radioactive materials in rocks and soil.
Geiger counters offer a straightforward solution for monitoring radiation with basic electronic components, making them indispensable tools in many professional and scientific applications.
Historical Use of Geiger Counters
In this 1963 photograph, a staff member from the Centers for Disease Control and Prevention (CDC) is seen using a Geiger-Müller counter, commonly known as a Geiger counter, to survey food items for potential fallout contamination. This image underscores the critical role of Geiger counters in public health and safety, especially during periods of nuclear tension when monitoring for radioactive contamination in consumables was essential.
Understanding Geiger Counters
Geiger counters are fundamental particle detectors that measure ionizing radiation, including various forms of nuclear radiation such as alpha particles, beta particles, and gamma rays. These devices work by ionizing gases within a Geiger-Müller tube, a process that generates a pulse of current for each detected particle, signaling the presence of radiation.
Key Features and Operation
- Ionization Process: Radiation particles ionize the gas inside the Geiger-Müller tube.
- Current Pulse: Each ionization event creates a pulse of current.
- Radiation Detection: The pulses are counted, providing a measure of the radiation present.
Applications and Importance
The simplicity and effectiveness of Geiger counters make them invaluable tools in various fields requiring radiation detection and safety assessments. Here are some key areas where Geiger counters are extensively used:
- Environmental Monitoring: Detecting radioactive contamination in air, water, and soil.
- Public Health: Ensuring consumables are free from harmful radiation levels, as demonstrated by the CDC’s historical use.
- Medical Field: Monitoring radiation exposure for patients and healthcare workers.
- Industrial Safety: Assessing radiation levels in nuclear plants and other industrial settings.
- Scientific Research: Conducting experiments involving radioactive materials.
Legacy and Continued Use
Since their invention, Geiger counters have been widely adopted across diverse sectors, trusted for their reliability in detecting ionizing radiation and for their straightforward, user-friendly operation. The historical application of Geiger counters highlights their essential role in protecting public health and ensuring safety by monitoring environments and consumables for radiation contamination.
For more information on ionizing radiation and its detection, visit Understanding Ionizing Radiation and Protection.
8. Whole Body Counters
Understanding Whole Body Counters
Whole body counters are sophisticated devices designed to measure the amount of radioactive substances within the human body. They are particularly useful for assessing exposure to gamma radiation, which cannot easily escape the body due to absorption or other interactions that cause the radiation to lose energy. This necessitates precise measurement technologies to accurately gauge internal exposure levels.
How Whole Body Counters Work
These counters operate by detecting gamma radiation emitted from radioactive decay within the body. Due to the varied nature of radiation distribution in the human body, whole body counters are designed to accommodate different positions, allowing measurements to be taken while an individual is sitting, lying down, or standing. This flexibility ensures that radiation measurements are not only accurate but also comfortable for the individual being assessed.
Configuration Options
Whole body counters can feature single or multiple detectors, which can be either stationary or mobile. The choice between these configurations depends on the specific requirements of the radiation assessment process:
- Stationary Systems: Typically used for routine screenings, these systems provide consistent and reliable measurements.
- Mobile Detectors: Offer more flexibility, allowing for detailed scans that can better identify the distribution and concentration of radioactive substances within the body.
Applications and Benefits
Whole body counters are crucial in various fields, including:
- Medical Diagnostics: Monitoring patients who have been exposed to radioactive substances to ensure their safety and guide treatment decisions.
- Occupational Safety: Assessing radiation exposure in workers who operate in environments with potential radioactive contamination.
- Environmental Monitoring: Checking for contamination in populations living near nuclear facilities or areas affected by radiological events.
By accurately measuring internal radiation levels, whole body counters help protect individuals from the potential health effects of radioactive exposure, ensuring compliance with safety standards and aiding in effective health management.
For more detailed information about the applications and technology of whole body counters, visit Whole Body Counters on ResearchGate.
Advantages and Challenges of Whole Body Counting
Whole body counting provides several significant benefits in radiation safety and health monitoring:
- Direct Measurement: Whole body counters measure the radionuclides present in the body directly, providing accurate assessments without relying on indirect methods such as urinalysis.
- Detection of Insoluble Radionuclides: This technique is particularly valuable for detecting insoluble radionuclides in the lungs that might not be identifiable through other methods.
- Straightforward Quantification: Whole body counting offers a direct and clear quantification of internal contamination, simplifying the process of determining radiation exposure.
However, whole body counting also presents several challenges:
- Limited to Gamma Emitters: The technique is most effective for gamma emitters, with other types of radiation being detectable only under specific conditions.
- Risk of Misinterpretation: External contamination can be mistakenly interpreted as internal contamination if proper decontamination procedures are not followed before measurement.
- Distinguishing Radioisotopes: Differentiating between radioisotopes with similar gamma energies can be challenging without additional data or analysis.
- Alpha and Beta Radiation Detection: Alpha and beta radiation are generally undetectable by external devices due to body shielding, though coincident gamma emissions from alpha decay and radiation from parent or daughter nuclides can sometimes be detected.
Calibration and Standardization
Radiation detection, including whole body counting, relies on relative measurements. These readings, typically in counts per minute or per second, must be calibrated against known standards to accurately determine the quantity of radioactive material present. Whole body counters achieve this calibration using a device known as a “phantom,” with the Bottle Manikin Absorber (BOMAB) phantom being the industry standard.
The BOMAB Phantom
The BOMAB phantom consists of 10 high-density polyethylene containers filled with a precisely known distribution and activity of radioactive material. This setup ensures the accuracy of the system in measuring high-energy photon emitters between 200 keV and 3 MeV.
Importance of Calibration
Calibration and standardization of in vivo counting systems, particularly using the BOMAB phantom, were underscored as critical needs at the 1990 international meeting of in vivo counting professionals at the National Institute of Standards and Technology (NIST). This meeting highlighted the necessity for consistent phantom specifications to ensure accurate and reliable measurements across various in vivo counting systems.
Why Calibration Matters
- Ensures Accuracy: Calibration against known standards ensures that the radiation measurements are accurate and reliable.
- Standardization: Using standardized phantoms like the BOMAB ensures consistency in measurements across different systems and settings.
- Compliance: Accurate calibration helps meet regulatory requirements and ensures safe levels of radiation exposure for individuals being monitored.
For further information, explore more about the BOMAB phantom and its uses in radiation measurement calibration at the National Institute of Standards and Technology (NIST) website.
Efficiency of Well-Designed Counting Systems
Well-designed counting systems excel in detecting low levels of gamma emitters (greater than 200 keV), which are far below the levels that could cause adverse health effects. For example, the typical detection limit for radioactive cesium (Cs-137) is around 40 Bq, whereas the Annual Limit on Intake (ALI)—the amount that would expose a person to a dose equivalent to the worker limit of 20 mSv—is approximately 2,000,000 Bq. These systems can also detect the naturally occurring radioactive potassium in humans, which is not harmful despite its presence in the body.
High Sensitivity and Low Background Radiation
The remarkable sensitivity of these instruments is largely due to their housing within low background counting chambers. These chambers are typically constructed as small rooms with walls about 20 cm thick, made of low-background steel. They may also be lined with approximately 1 cm of lead to further reduce background radiation. The reduction achieved within these chambers can be several orders of magnitude, significantly enhancing the sensitivity of the counting systems.
Utilization of Low-Background Steel
Some counting systems use historically low-background steel for additional shielding—such as armor plating sourced from pre-nuclear era warships. This material helps shield against contemporary environmental radiation, further improving the system’s accuracy and sensitivity.
Geometry and Count Times
The geometry of the counting system affects the count times, which can range from 1 minute to about 30 minutes. The sensitivity of these counters depends on the counting time; generally, longer counts within the same system result in better detection limits, allowing for more precise and reliable measurements of radioactive substances.
Benefits of Well-Designed Systems
- Detection of Low Levels: Capable of identifying low levels of gamma emitters well below harmful thresholds.
- High Sensitivity: Enhanced by low background radiation chambers and historical low-background steel.
- Adjustable Count Times: Longer count times improve detection limits, ensuring accurate measurements.
These features make well-designed counting systems indispensable in radiation safety and health monitoring, providing accurate and reliable data essential for assessing exposure levels and ensuring compliance with safety standards.
For more detailed information on radiation detection and safety, visit the National Institute of Standards and Technology (NIST) website.
9. Biological Survey
Cytogenetic Biodosimetry: Estimating Radiation Exposure through Biological Responses
Cytogenetic biodosimetry is a powerful method that uses the body’s biological response to radiation to accurately estimate exposure levels. This technique is based on the principle that ionizing radiation, when absorbed by the human body, transfers energy to cells and tissues, causing chromosomal DNA damage. This damage occurs in proportion to both the intensity and type of radiation absorbed.
Measuring Chromosomal Abnormalities
Radiation exposure induces chromosomal abnormalities such as breaks and rearrangements. In cytogenetic biodosimetry, these abnormalities are carefully measured and analyzed. The process involves comparing the frequency and severity of chromosomal abnormalities against a calibration curve, which is established through extensive research. This comparison allows scientists and healthcare providers to accurately estimate the radiation dose a person has received.
Reliability across Different Conditions
One of the key strengths of cytogenetic biodosimetry is its reliability across different conditions. Whether lymphocytes—the type of white blood cells that exhibit chromosomal damage—are inside or outside the body at the time of radiation exposure, they consistently express the extent of chromosomal damage accurately. This makes cytogenetic biodosimetry an essential tool in both medical diagnostics and radiation emergency response.
Applications in Medical Diagnostics and Emergency Response
- Medical Diagnostics: Provides critical data for diagnosing radiation exposure and guiding treatment decisions.
- Radiation Emergency Response: Offers essential information for health monitoring strategies and emergency response planning.
Cytogenetic biodosimetry plays a crucial role in ensuring accurate radiation exposure assessments. By leveraging the body’s natural biological responses, this technique helps safeguard public health and enhances the effectiveness of medical and emergency interventions.
For more information on radiation detection and safety, visit the National Institute of Standards and Technology (NIST) website.
Cytogenetic Biodosimetry for Radiation Exposure Assessment
Ionizing radiation can cause significant damage to human cells and tissues at the molecular level, particularly affecting chromosomal DNA. This damage is directly proportional to both the type and amount of radiation energy absorbed by the body. Cytogenetic biodosimetry employs human peripheral blood lymphocytes (HPBLs) to detect and quantify this damage. Among the chromosomal abnormalities induced by radiation exposure, dicentric chromosomes are a common and highly indicative marker.
Quantifying Dicentric Chromosomes
Cytogenetic biodosimetry involves meticulous quantification of dicentric chromosomes within HPBLs. The frequency of these abnormalities is compared against a pre-established calibration curve to estimate the radiation dose received. This method’s validity is reinforced by the consistent manifestation of radiation-induced chromosomal damage in lymphocytes, regardless of their location in the body.
Sample Collection and Analysis
- Blood samples (typically less than 10 mL) are collected from individuals suspected of radiation exposure.
- Samples should be collected as soon as possible, ideally within a day of the incident.
- Samples are transported to specialized cytogenetic biodosimetry laboratories for detailed examination.
- Trained professionals process and analyze the samples using rigorous protocols to assess cytogenetic damage.
The dose assessment is conducted by comparing the observed genetic damage with calibration curves. These curves account for variables such as the type of radiation, dose rate, whole or partial body exposure, the time elapsed between exposure and sample collection, and the specific methods used for cytogenetic analysis.
Applications in Radiation Emergencies and Occupational Exposure
Cytogenetic biodosimetry is crucial when physical dosimetry tools are unavailable or infeasible. It has proven invaluable in various radiation emergencies globally, including notable incidents at Chernobyl, Goiânia, and Tokaimura. Beyond emergency responses, cytogenetic analysis is routinely used to investigate suspected occupational overexposures, providing essential data to confirm or rule out significant radiological exposure.
For more information on managing and assessing radiation exposure in occupational settings, visit our detailed guide on Occupational Radiation Exposure Limits.
The Baby Tooth Survey: Tracing Nuclear Fallout in Children’s Teeth
The Baby Tooth Survey, initiated by the Greater St. Louis Citizens’ Committee for Nuclear Information in collaboration with Saint Louis University and the Washington University School of Dental Medicine, aimed to assess the impact of nuclear fallout on human health. This pioneering research project focused on measuring the accumulation of strontium-90—a carcinogenic radioactive isotope produced by atmospheric nuclear tests—in the deciduous teeth of children. Strontium-90, due to its chemical similarity to calcium, is readily absorbed from water and dairy products into bones and teeth.
Collecting Baby Teeth for Research
The survey team distributed collection forms to schools across the St. Louis, Missouri area, with an ambitious goal to collect 50,000 teeth annually. By the project’s conclusion in 1970, over 300,000 teeth had been collected, providing a vast dataset for analysis.
- **Key Findings:** Early findings, published in the November 24, 1961 issue of Science, indicated a troubling trend: strontium-90 levels in children born during the 1950s had steadily increased, with those born later exhibiting significantly higher concentrations.
- **Significant Increase:** This upward trend was particularly stark in children born after 1963, who had strontium-90 levels in their teeth that were 50 times higher than those in children born before large-scale nuclear testing began.
Influencing Policy and Global Impact
The profound impact of the Baby Tooth Survey’s findings resonated at the highest levels of international policy. The data collected were instrumental in convincing U.S. President John F. Kennedy of the urgent need for a nuclear test ban, leading him to champion and ultimately sign the Partial Nuclear Test Ban Treaty alongside the United Kingdom and Soviet Union. This landmark treaty significantly curtailed above-ground nuclear weapons testing, thereby mitigating the release of nuclear fallout into the environment and marking a crucial step in global nuclear disarmament efforts.
The success and methodology of the Baby Tooth Survey also set a precedent for similar research initiatives worldwide, illustrating the power of scientific inquiry in influencing public health and policy.
Global Legacy and Continued Influence
The legacy of the Baby Tooth Survey continues to underscore the importance of scientific research in shaping public health initiatives and forging international health and environmental standards. A prime example of such influence is the Tooth Fairy Project in South Africa, established to explore the potential health impacts of radioactivity and heavy metal contamination linked to acid mine drainage from extensive gold mining.
These investigations are critical as they provide valuable insights into the environmental impacts of industrial activities and inform strategies for mitigating these impacts in affected communities.
Learn more about the impact of the Baby Tooth Survey and its methodologies in shaping public health and environmental policies through similar initiatives worldwide at Reducing Radiation Exposure in Digital Imaging.
10. Kearny Fallout Meter
The Kearny Fallout Meter (KFM) is a simple yet effective radiation meter that can be constructed using everyday materials. This device was designed to enable individuals to measure fallout radiation levels following a nuclear event using commonly available items. The KFM can be made from a coffee can or pail, a small amount of gypsum board, monofilament fishing line, and aluminum foil.
How the Kearny Fallout Meter Works
The operational principle of the KFM involves the use of electrostatic principles to detect ionizing radiation:
– **Aluminum Foil Strips:** Inside the can, two strips of aluminum foil serve as leaves.
– **Electrostatic Charge:** An electrostatic charge is applied to these leaves; since they carry the same type of charge, they repel each other.
– **Ionization and Measurement:** Radiation within the can ionizes the air, altering the electric charge and affecting the degree of repulsion between the two foil strips. The extent of their repulsion is then used to gauge the level of radiation in the surrounding area.
Building a Kearny Fallout Meter
Comprehensive plans for building a Kearny Fallout Meter are available and can be accessed at this link. This DIY approach not only makes radiation measurement accessible but also serves as an educational science project that demonstrates the effects of ionizing radiation in a tangible way.
“The KFM was developed by Cresson Kearny based on research conducted at Oak Ridge National Laboratory.”
Historical Context and Development
The KFM was developed by Cresson Kearny, who based his work on research conducted at Oak Ridge National Laboratory. His efforts are detailed in the civil defense manual “Nuclear War Survival Skills,” which aimed to provide the public with practical survival techniques and tools in the event of nuclear fallout, emphasizing self-reliance and preparedness.
For more detailed instructions on how to build a Kearny Fallout Meter, refer to the original document by Cresson Kearny at the provided link. This resource outlines the step-by-step construction process and the science behind the device, making it a valuable tool for understanding and preparing for radiation exposure.
FAQs
1. What is a Geiger-Muller counter and how does it function?
A Geiger-Muller counter is a device used to detect ionizing radiation. It functions by using a Geiger-Muller tube filled with gas. When ionizing radiation passes through the tube, it ionizes the gas, producing an electrical pulse that is counted and displayed as a measure of radiation intensity.
2. How do scintillation detectors detect radiation?
Scintillation detectors detect radiation by using a scintillating material that emits light when struck by ionizing radiation. This emitted light is then captured and amplified by a photomultiplier tube, which converts it into an electrical signal proportional to the radiation intensity.
3. What is the purpose of an ionization chamber in radiation detection?
An ionization chamber is used to measure radiation exposure by collecting ions produced by ionizing radiation in a gas-filled chamber. The collected ions generate a current that is directly proportional to the amount of radiation, allowing for accurate measurement of radiation dose.
4. What are dosimeters and how are they used in radiation monitoring?
Dosimeters are personal radiation monitoring devices used to measure the cumulative radiation dose received by an individual over time. They are essential for ensuring that radiation workers do not exceed safe exposure limits. Dosimeters can be worn as badges or rings and provide critical safety information.
5. How do neutron detectors operate differently from other radiation detectors?
Neutron detectors are specifically designed to detect neutrons, which are neutral particles that do not ionize directly. These detectors often use materials that undergo nuclear reactions with neutrons, producing secondary charged particles that can be detected and measured to determine neutron radiation levels.